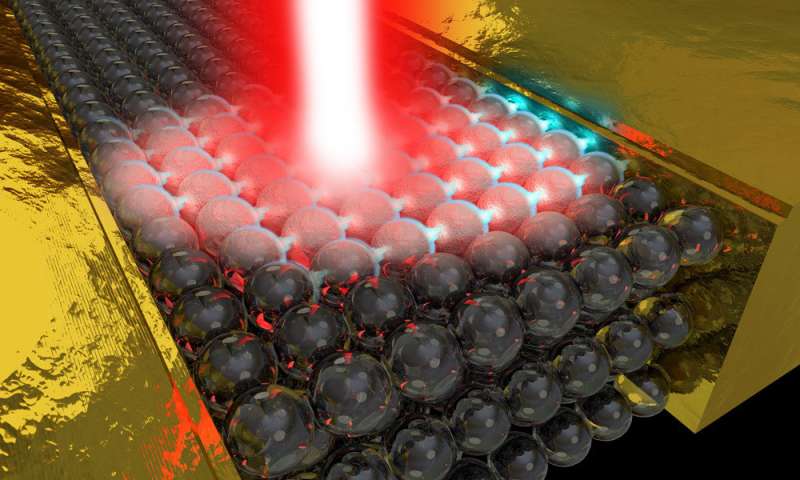
Take a glass thread a thousand times thinner than a human hair. Use it as a wire between two metals. Hit it with a laser pulse that lasts a millionth of a billionth of a second.
Remarkable things happen.
The glass-like material is transformed ever so briefly into something akin to a metal. And the laser generates a burst of electrical current across this tiny electrical circuit. It does so far faster than any traditional way of producing electricity and in the absence of an applied voltage. Further, the direction and magnitude of the current can be controlled simply by varying the shape of the laser—by changing its phase.
Now a University of Rochester researcher—who predicted laser pulses could generate ultrafast currents along nanoscale junctions like this in theory—believes he can explain exactly how and why scientists succeeded in creating these currents in actual experiments.
“This marks a new frontier in the control of electrons using lasers,” says Ignacio Franco, assistant professor of chemistry and physics. He has collaborated with Liping Chen, a postdoctoral associate in his group, and with Yu Zhang and GuanHua Chen at the University of Hong Kong on a computational model to recreate and clarify what happened in the experiment. This work funded by Franco’s NSF CAREER award is now published in Nature Communications.
“You will not build a car out of this, but you will be able to generate currents faster than ever before,” Franco says. “You will be able to develop electronic circuits a few billionths of a meter long [nanoscale] that operate in a millionth of a billionth of a second [femtosecond] time scale. But, more importantly, this is a wonderful example of how differently matter can behave when driven far from equilibrium. The lasers shake the nanojunction so hard that it completely changes its properties. This implies that we can use light to tune the behavior of matter.”
This is exactly what the US Department of Energy had in mind when it listed the control of matter at the level of electrons—and understanding matter “very far away” from equilibrium—among its key challenges for the nation’s scientists.
The DOE issued those challenges in 2007. That same year, Franco, then a doctoral student at the University of Toronto, was lead author of a paper in Physical Review Letters theorizing that extremely powerful, ultrafast electrical currents could be generated in molecular wires exposed to femtosecond laser pulses.
The molecular wires, made of a linear carbon chain, would be connected to metallic contacts forming a nanoscale junction. The current would be generated because a phenomenon called the Stark effect, in which the energy levels of matter are shifted due to the presence of the external electric field of the laser, is used to control level alignment between the molecule and the metallic contacts.
But this theoretical proposal remained just that. The challenges of actually building a junction that small, and then being able to document what happened before the wires were destroyed by the lasers, were too daunting to validate the theory with actual experiments.
That is until 2013, when researchers led by Ferenc Krausz at the Max Planck Institute of Quantum Optics were able to generate ultrafast currents by exposing a different nanojunction—glass connecting two gold electrodes—to laser pulses.
The exact dynamics involved remained unclear, Franco says. Various theories were advanced by other researchers. But even though the materials were different, Franco suspected involvement of the same Stark effect mechanisms hypothesized in his 2007 paper.
A four-year simulation effort, involving millions of computing hours of Blue Hive computer processing, have confirmed that, says Franco. “We were able to recover the main experimental observations using state-of-the-art computational methods, and develop a very simple picture of the mechanism behind the experimental observations,” he says.
The research illustrates how theory and experiment are mutually reinforcing in advancing science, Franco says. “Theory led to an experiment that nobody really understood, resulting in better theories that are now leading to better experiments” he says. “This is an area in which we still have a lot of things to understand,” he adds.
Chemists have traditionally studied the relationship of a molecule’s structure to its possible functions when the material is at or near thermodynamic equilibrium, he says.
“This research invites you to think about structure-function relations that apply very, very far away from equilibrium.”